STUDY OF THE SYNTHESIS OF A SENSOR MATERIAL BASED ON THE OXIDES ZICCONIUM/EUROPIUM
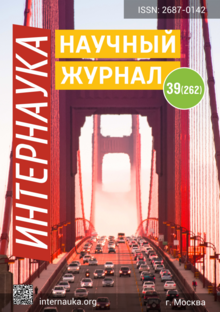
STUDY OF THE SYNTHESIS OF A SENSOR MATERIAL BASED ON THE OXIDES ZICCONIUM/EUROPIUM
Ngo Quang Huy
M.S., Institute for Technology of Radioactive and Rare elements,
Vietnam, Ha Noi
Nguyen Dinh Viet
M.S., Institute for Technology of Radioactive and Rare elements,
Vietnam, Ha Noi
Nguyen Thanh Thuy
M.S., Institute for Technology of Radioactive and Rare elements,
Vietnam, Ha Noi
Luu Xuan Dinh
Ph.D, Institute for Technology of Radioactive and Rare elements,
Vietnam, Ha Noi
Bui Cong Trinh
Ph.D, Institute for Technology of Radioactive and Rare elements,
Vietnam, Ha Noi
Acknowledgments: This research was financially supported by the project of VINATOM (code: CS/22/03-02)
ABSTRACT
Due to its high sensitivity and selectivity, vapor tracing of volatile organic molecules based on photocatalytic luminescence is attracting attention. The base material, metal oxide, is combined with rare earth elements to increase luminescence. In this work, zirconium oxide nano-based sensor materials with additional europium oxide were synthesized in a wet method in two stages: hydroxide precipitation by an exchange reaction between their salts with NH4OH and oxide creation via calcining in air. The precipitation stage showed that the following conditions: % mol Eu/Zr 5%, pH = 8, stirring speed 200 rpm, reaction duration 2 hours, will produce a precipitate with a yield of 95-96 % and particle size of 1-5 µm. The calcination process in air, the specific surface area of the sample reaches 84,40 m2/g, the particle size is about 30-32 nm at 600oC, and the calcination time is 2 hours.
Keywords: Cataluminescence, sensor, ziconium, europium.
I. Introduction
The topic of vapor traceability of low concentrations of volatile organic compounds (VOCs) has attracted more attention in recent years. VOC detection is critical in a variety of fields, including environmental monitoring [1,2], medical diagnostics [3-5], air quality monitoring [6-8], and national security rooms [9]. Because of the growing threat of international terrorism, the detection of low quantities (in the ppb range) of explosive vapors in the air is of increasing importance in the national security industry [10-12].
One method for tracing VOCs is based on the leading chemical sensing technology, specifically the transition metal oxide gas sensor [13-15]. The detection mechanism of changing the resistance of materials has excellent sensitivity, but the restriction is limited selectivity. The most common materials investigated include oxides of yttrium, zinc, vonfram, titanium, and zirconium [16-21].
Cataluminescence (CTL) is another feature of the less widely utilized metal oxide-based subgroups [22]. In a heterogeneous catalytic oxidation reaction, luminescence catalyst produces chemiluminescence on the surface of a solid catalyst. CTL has several advantages, including high sensitivity, quick reaction, minimal equipment, and long-term stability. TiO2, Al2O3, ZrO2, and other substrates are commonly utilized in CTL processes. Many investigations have demonstrated that nano-sized zirconium materials play a catalytic role in the conversion of various organic molecules such as ethanol, acetone, 2,4-DND, DMNB...[23]. When a heterogeneous catalytic process of vaporization of organic compounds on the solid phase surface occurs, adding Europium to Zirconium nanoparticles increases fluorescence emission.
II. Experiment
II.1. Method of materials synthesis
According to studies on the synthesis of mixed oxide nanomaterials [24–26], the wet method is still utilised for systems based on Al2O3, TiO2, ZrO2... with mixed rare earth elements primarily due to the quicker synthesis time than the sol gel method, while still guaranteeing the uniform distribution of the components in the synthesized sample.
The stages involved in using the wet technique to synthesize materials are as follows: In the combined solution of ZrO(NO3)2 and Eu(NO3)3, add an equivalent amount of NH4OH solution (according to reactions (1) and (2)). The mixture was thoroughly swirled throughout the reaction. The white precipitate underwent filtering and numerous rounds of deionized water washing. Following that, the sample was dried at a temperature of 120oC in an oven. After drying, the sample is heated to a high temperature in a stationary furnace in the final stage of synthesis.
The following are the main precipitation reactions:
ZrO(NO3)2 + 2NH4OH = ZrO(OH)2 + 2NH4NO3 (1)
Eu(NO3)3 + 3NH4OH = Eu(OH)3 + 3NH4NO3 (2)
The main reactions that occur during calcination are as follows:
ZrO(OH)2 = ZrO2 + H2O (3)
2Eu(OH)3 = Eu2O3 + 3H2O (4)
Figure 1. Schematic of material synthesis
II.2. Chemicals and equipment
Chemicals: Chinese PA products with purity levels of > 99.9% for NH4OH, ZrO(NO3)2 and Eu(NO3)3.
Equipments: Volumetric flasks in sizes of 500 and 1000 ml. 100, 200, 500, and 1000ml measuring tubes are used. Filter paper, a porcelain basin, and a funnel.
Equipment includes a pH meter, a drying oven, a furnace, and an analytical balance.
III. Results and Discussion
The team's main goal in this investigation was to examine the material synthesis process's parameters. The Zr/Eu molar ratio, pH, and stirring speed are the variables that need to be looked into for the precipitation process in order to get the best precipitation efficiency.
The team concentrated on researching the heating temperature and time for the precipitation sintering process to produce the product with the greatest specific surface area.Subsequent pu blications give experimental findings to assess the capability to detect (luminescence) in ethanol vapor.
III.1. Process of precipitation
III.1.a. Effect of the Zr/Eu molar ratio
Conditions for the experiment are as follows: pH = 8, 2 hours of stirring at 200 rpm. ZrO(NO3)2 and Eu(NO3)3 solutions had fixed volumes of 10 and 2.5 ml, respectively. Their concentrations were adjusted to produce a Eu/Zr ratio that ranged from 1% to 10%. (range investigated in studies [24-26] with homologous materials).
Table 1.
The ratio of Eu/Zr moles in the studied samples
C(ZrO(NO3)2), (M) |
C(Eu(NO3)2), (M) |
n(Zr), (mol) |
n(Eu), (mol) |
n(Eu/Zr), (%) |
0,3 |
0,02 |
0,003 |
0,00005 |
1,7 |
0,2 |
0,02 |
0,002 |
0,00005 |
2,5 |
0,1 |
0,02 |
0,001 |
0,00005 |
5,0 |
0,075 |
0,02 |
0,00075 |
0,00005 |
6,7 |
0,05 |
0,02 |
0,0005 |
0,00005 |
10,0 |
The precipitate was dried at 120oC for 3 hours, determined the precipitate formation efficiency and taken for SEM.
Table 2.
Precipitation efficiency of samples at different molar ratios of Eu/Zr
n(Eu/Zr), (%) |
H, (%) |
1,7 |
96,4 |
2,5 |
95,4 |
5,0 |
96,5 |
6,7 |
94,8 |
10 |
96,7 |
The effectiveness of the samples at forming precipitation is not significantly different, as shown in Table 2. No sample with 100% efficiency was found in the survey samples.
Figure 2. SEM images of samples with different Eu/Zr ratios
The similarity in surface morphology of the acquired samples may be shown in Figure 2. The samples are not lumpy, and the particle distribution is rather homogeneous. Particle size from 1 and 5 µm.
III.1.b. Effect of stirring speed
Conditions for the experiment are as follows: 2 hours for a reaction at pH = 8. Use of 10 ml of 0,1 M ZrO(NO3)2 and 2,5 ml of 0,02 M Eu resulted in a 5% mole ratio of Eu/Zr.
Investigations into the stirring speed were done at 100, 150, 200, and 300 rpm. Localized clumping in the response system was seen at speeds lower than 100 rpm. A significant volume of substance is blasted on the wall or spills out at speeds greater than 300 rpm.
Table 3.
Precipitation efficiency at different stirring speeds
Speed, rpm |
H, (%) |
100 |
96,7 |
150 |
95,9 |
200 |
96,7 |
300 |
94,3 |
The effectiveness of precipitation synthesis is high, above 94%, similar to the study with Eu/Zr ratios. Additionally, there was no discernible linear change in efficiency with stirring speed.
Figure 3. SEM images of samples at different stirring modes
With a speed of under 300 rpm, it is evident from Figure 3 that the particle distribution is comparatively uniform. High stirring rates, however, cause a concentrated dispersion to be seen in specific matrix locations. The following experiment was carried out at a 200 rpm stirring speed.
III.1.c. Effect of pH
Conditions for the experiment are as follows: The reaction mixture of 10 ml of 0,1 M ZrO(NO3)2 and 2,5 ml of 0,02 M Eu(NO3)3 is rapidly added ammonia over the course of two hours (equivalent to 0,02 M). 200 rpm stirring speed, 5% mole Eu/Zr.
pH levels at 8, 9, 10, and 11 were investigated. 0,1 M of NaOH solution was used to alter pH.
Table 4.
Precipitation efficiency at different pH
pH |
8 |
9 |
10 |
11 |
H, (%) |
95,7 |
96,9 |
95,7 |
94,8 |
The efficacy of the samples at generating precipitation is not significantly different, as shown in Table 4. No sample with 100% efficiency was found in the examined samples, which can be explained by precipitate loss during filtration and washing.
Figure 4. SEM images of samples obtained at different pH conditions
The particles have an oval shape, are distributed quite uniformly, and exhibit no clumping at resolutions up to 500 nm. This consistent distribution will aid in the production of smaller grain sizes during sintering because the particles must go through high-temperature calcination.
III.2. Calcination process
III.2.a. Effect of temperature
The filming time is set at four hours. At temperatures of 400, 500, 600, and 700oC, the calcination temperature was examined. 15oC per minute of heating occurs. Following calcination, the sample was allowed to cool in the air, and the specific surface area was calculated using BET and base particle size measurements (via TEM).
Table 5.
Surface morphology of samples at different temperatures
T, 0C |
400 |
500 |
600 |
700 |
S, m2/g |
60,62 |
82,46 |
82,75 |
83,23 |
Particle size, nm |
200 |
68 |
30 |
32 |
Table 5 shows that the sample's specific surface area changes dramatically as the heating temperature is raised. Below 500oC, the dehydration reaction only partially takes place. The base particle size reached 30-32 nm at 600 and 700oC, and the specific surface area was at its maximum. The firing temperature for the experiment to study the firing time was chosen to be 600oC.
III.2.b. Effect of heating time
At 600oC, the calcination temperature was set. 15oC per minute of heating occurs. 1, 2, 3, and 4 hours were investigated for the calcination time..
Table 6.
Specific surface area of samples at different calcination temperatures
Time, h |
1 |
2 |
3 |
4 |
S, m2/g |
68,43 |
84,40 |
83,97 |
84,32 |
Particle size, nm |
110 |
33 |
35 |
32 |
No linear increase in the specific surface area with calcination time was seen in Table 6. As the calcination duration rose from 1 hour to 2 hours throughout the first 2 hours, the sample's specific surface area gradually increased from 68,43 to 84,40 m2/g. After two hours, the sample's specific surface area rose and nearly reached 84,32 m2/g, whereas the base particle size remained constant and held true between 32 and 35 nm.
IV. Conclusion
In this study, the group used a wet approach with two steps — hydroxide precipitation and oxide formation — to produce europium oxide nanomaterials on a base of zirconium oxide. It was discovered that the nitrate salts of Eu and Zr easily exchanged with NH4OH during precipitation to create the metal hydroxides. When utilizing the ratio Eu/Zr 5%mol, stirring speed 200 rpm, pH 8, and two-hour reaction times, precipitation efficiency was 95–96%. The particles were evenly distributed and their average size was 1 to 5 µm after drying at 120oC. The analysis of the oxide-generating process revealed the ideal calcination mode parameters: calcination duration of 2 hours for ZrO2/Eu2O3 products with specific surface area emitted, heating temperature of 600oC, heating rate of 15oC/min. increase until they reach 84,40 m2/g, with particles between 30 and 32 nm in size.
References:
- M.S. Kamal, S.A. Razzak, M.M. Hossain, Catalytic oxidation of volatile organic compounds (VOCs) - a review, Atmos. Environ.
- J.E. Colman Lerner, M. de los, A. Gutierrez, D. Mellado, D. Giuliani, L. Massolo, E. Y. Sanchez, A. Porta, Characterization and cancer risk assessment of VOCs in home and school environments in gran La Plata, Argentina, Environ. Sci. Pollut. Res. (2018).
- A.D. Wilson, Application of electronic-nose technologies and VOC-biomarkers for the noninvasive early diagnosis of gastrointestinal diseases, Sensors (Switzerland). (2018).
- A.M. Casas-Ferreira, M. del Nogal-S´ anchez, J.L. P´erez-Pavon, ´ B. Moreno-Cordero, Non-separative mass spectrometry methods for non-invasive medical diagnostics based on volatile organic compounds: a review, Anal. Chim. Acta .(2019).
- A. Pizzini, W. Filipiak, J. Wille, C. Ager, H. Wiesenhofer, R. Kubinec, J. Blaˇsko, C. Tschurtschenthaler, C.A. Mayhew, G. Weiss, R. Bellmann-Weiler, Analysis of volatile organic compounds in the breath of patients with stable or acute exacerbation of chronic obstructive pulmonary disease, J. Breath Res. (2018).
- C.S. Lee, Z. Dai, D.H. Kim, H.Y. Li, Y.M. Jo, B.Y. Kim, H.G. Byun, I. Hwang, J. H. Lee, Highly discriminative and sensitive detection of volatile organic compounds for monitoring indoor air quality using pure and Au-loaded 2D In2O3 inverse opal thin films, Sensors Actuators, B Chem. (2018).
- P. Arroyo, J.L. Herrero, J.I. Suarez, ´ J. Lozano, Wireless sensor network combined with cloud computing for air quality monitoring, Sensors (Switzerland). (2019).
- M.F. Yassin, A.M. Pillai, Monitoring of volatile organic compounds in different schools: a determinant of the indoor air quality, Int. J. Environ. Sci. Technol. (2019).
- S. Oztürk, ¨ A. Kosemen, ¨ Z. S¸ en, N. Kılınç, M. Harbeck, Poly(3-methylthiophene) thin films deposited electrochemically on QCMs for the sensing of volatile organic compounds, Sensors (Switzerland). (2016).
- C. Tudisco, A. Motta, T. Barboza, C. Massera, A.E. Giuffrida, R. Pinalli, E. Dalcanale, G.G. Condorelli, Cavitand-decorated silicon columnar nanostructures for the surface recognition of volatile nitroaromatic compounds, ACS Omega (2018).
- J.R. Askim, Z. Li, M.K. LaGasse, J.M. Rankin, K.S. Suslick, An optoelectronic nose for identification of explosives, Chem. Sci. (2016).
- K. Konstantynovski, G. Njio, F. Borner, A. Lepcha, T. Fischer, G. Holl, S. Mathur, Bulk detection of explosives and development of customized metal oxide semiconductor gas sensors for the identification of energetic materials, Sensors Actuators, B Chem. (2018).
- A. Dey, Semiconductor metal oxide gas sensors: a review, Mater. Sci. Eng. B SolidState Mater. Adv. Technol. (2018).
- T. Lin, X. Lv, Z. Hu, A. Xu, C. Feng, Semiconductor metal oxides as chemoresistive sensors for detecting volatile organic compounds, Sensors (Switzerland). (2019).
- D. Nunes, A. Pimentel, A. Goncalves, S. Pereira, R. Branquinho, P. Barquinha, E. Fortunato, R. Martins, Metal oxide nanostructures for sensor applications, Semicond. Sci. Technol. (2019).
- A. Staerz, T. Russ, U. Weimar, N. Barsan, Understanding the sensing mechanism of WO3 based gas sensors, ISOEN 2019 - 18th Int. Symp. Olfaction Electron. Nose, Proc. (2019).
- J. Zheng, T. Zhang, H. Zeng, W. Guo, B. Zhao, Y. Sun, Y. Li, L. Jiang, Multishelled hollow structures of yttrium oxide for the highly selective and ultrasensitive detection of methanol, Small. (2019).
- B.P.J. de Lacy Costello, R.J. Ewen, N.M. Ratcliffe, M. Richards, Highly sensitive room temperature sensors based on the UV-LED activation of zinc oxide nanoparticles, Sensors Actuators, B Chem. (2008).
- L.E. Ocola, Y. Wang, R. Divan, J. Chen, Multifunctional UV and gas sensors based on vertically nanostructured zinc oxide: volume versus surface effect, Sensors (Switzerland). (2019).
- B. Gokdere, A. Üzer, S. Durmazel, E. Erçag, R. Apak, Titanium dioxide nanoparticles–based colorimetric sensors for determination of hydrogen peroxide and triacetone triperoxide (TATP), Talanta. (2019).
- U. Guth, H.D. Wiemhofer, ¨ Gas sensors based on oxygen ion conducting metal oxides, in: gas sensors based conduct. met. oxides basic understanding, Technol. Appl. (2018).
- J. Hu, L. Zhang, Y. Lv, Recent advances in cataluminescence gas sensor: materials and methodologies, Appl. Spectrosc. Rev. (2019).
- Matteo Fois a, Timothy Cox b, Norman Ratcliffe a, Ben de Lacy Costello a. Rare earth doped metal oxide sensor for the multimodal detection of volatile organic compounds (VOCs). Sensors & Actuators: B. Chemical 330 , p. 129-264. (2021).
- Nakagawa, M., and Yamashita, N. Cataluminescence-based gas sensors.Frontiers in Chemical Sensors3: 93–132. doi: 10.1007/3-540-27757-9_3. (2005).
- Okabayashi, T., Fujimoto, T., Yamamoto, I., Utsunomiya, K., Wada, T., Yamashita, Y., Yamashita, N., and Nakagawa, M. High sensitive hydrocarbon gas sensor utilizing cataluminescence of g-Al2O3, activated with Dy 3C . Sens. Actuators, B64(1–3): 54–58. doi: 10.1016/S0925-4005(99) 00483-9. (2000).
- Zhu, Y. F., Shi, J. J., Zhang, Z. Y., Zhang, C., and Zhang, X. R. Development of a gas sensor utilizing chemiluminescence on nanosized titanium dioxide.Anal. Chem.74(1): 120–124. doi: 10.1021/ac010450p. (2002).